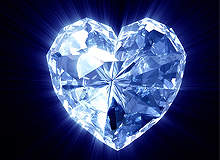
Since JC Bokros et al demonstrated the thromboresistance of pyrolytic carbon in 1969, carbon-based materials have been commonly used in the fabrication of heart valves and other medical devices for cardiovascular applications. Recently, significant research advances have been made in the processing and use of diamond as a medical device material.
In diamond, carbon atoms form hybridised bonds with a tetrahedral (sp3) geometry. Lonsdaleite is a rare polytype of diamond that exhibits a hexagonal structure; however, most naturally occurring diamond crystals exhibit what is known as the diamond cubic crystal structure, which GN Ramachandran described as consisting of two close-packed interpenetrating face-centred cubic lattices.
Due to the short carbon-carbon distance, the atomic density of the diamond crystal is the highest of any solid, while covalent-bonded materials such as diamond demonstrate greater bond strength than ionic or metallic-bonded materials, not only due to electron sharing but also because of high periodicity. As a result of this unusual structure, diamond exhibits interesting properties that are suitable for many medical device applications.
For example, it has the highest hardness (10,400kg per mm²) and highest bulk modulus (1,250GPa) of any material. In addition, it has an extremely low coefficient of friction; 0.05 in air, which is similar to that of polytetrafluoroethylene. It displays excellent wear resistance and corrosion resistance. Diamond also exhibits the highest known value of thermal conductivity at room temperature, as well as optical transparency from the ultraviolet to the infrared region of the electromagnetic spectrum.
From graphite to diamond
The graphite-to-diamond transformation under thermodynamic equilibrium conditions requires high pressures and high temperatures (HP-HT). An HP-HT technique for converting graphite into diamond was developed by William Eversole at the Union Carbide Company and FP Bundy et al at the General Electric Company in the early 1950s. HP-HT techniques produce small crystals, which are typically used for grinding, polishing and cutting, while low-pressure chemical vapour deposition techniques may be used to grow diamond coatings from an activated gas phase containing hydrogen (~99%) and a carbon-containing gas (such as methane); activation typically involves use of plasma, combustion flame or hot filament.
How well do you really know your competitors?
Access the most comprehensive Company Profiles on the market, powered by GlobalData. Save hours of research. Gain competitive edge.
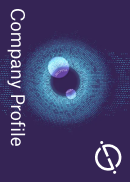
Thank you!
Your download email will arrive shortly
Not ready to buy yet? Download a free sample
We are confident about the unique quality of our Company Profiles. However, we want you to make the most beneficial decision for your business, so we offer a free sample that you can download by submitting the below form
By GlobalDataActivated hydrocarbon species decompose to generate excited carbon atoms, which form the diamond coating. According to D Zhou et al and S Michaelson et al, hydrogen is involved in terminating carbon dangling bonds, etching trigonally bonded carbon atoms from the coating surface, stabilising diamond clusters and abstracting hydrogen from surface carbon-hydrogen bonds.
Chemical vapour deposition methods have several benefits, including the ability to coat recesses and other 3D surfaces, high coating growth rates and the capability for co-deposition of two or more materials. Drawbacks include the use of potentially explosive gases and high processing temperatures, which preclude coating of polymers. Recently, physical vapour deposition methods, including laser irradiation and molecular beam techniques, have also been demonstrated.
Diamond’s medical role
Whatever the method, diamond coatings are applied to metallic or ceramic materials used in medical devices to minimise corrosion, wear and metal ion release. The chemical reactivity of the substrate material plays a major role in determining the diamond coating structure.
Growth of diamond coatings on non-diamond surfaces commonly involves initial growth of an interlayer material or mechanical manipulation (such as scratching) of the substrate, which promotes nucleation of diamond. However, growth of diamond coatings on several transition metals (such as cobalt, ferrous and nickel alloys) is difficult because of the high rate of carbon diffusion within these materials, which hinders nucleation of diamond, although bond formation with trigonal hybridisation and formation of graphite are promoted on the surfaces of elements with partially filled electron shells (copper).
In addition, a mismatch between the coefficients of thermal expansion of the bulk material and the diamond coating can lead to compressive stresses in the diamond coating, and delamination of the diamond coating may occur when cooling the material from the processing temperature to room temperature.
Interlayers between the diamond coating and the bulk material, including tungsten carbide, aluminum nitride, carbon nitride, titanium carbide and titanium nitride, may be used to minimise carbon diffusion and/or thermal stresses.
Development research
Significant research efforts have been undertaken to develop nanocrystalline diamond coatings, whereby nanocrystalline diamond is defined as materials with grain sizes smaller than 100nm, and ultrananocrystalline diamond is defined as materials with grain sizes smaller than 10nm.
O Auciello et al used a process involving carbon-containing noble gas plasmas (99% Ar and 1% CH4) and substrate temperatures 350-800°C to produce carbon dimers from methane.
They obtained nanocrystalline diamond coatings containing randomly oriented 3-15nm diamond crystallites – carbon atoms in grain boundaries exhibit p bonding – and possessing a much larger fraction of atoms at grain boundaries than microcrystalline diamond coatings.
DM Gruen suggests that these carbon atoms located at grain boundaries play a significant role in determining the mechanical behaviour of nanocrystalline diamond.
On a nanocrystalline diamond film deposited by means of microwave plasma-enhanced chemical vapour deposition on a p-type Si (100) wafer, the coating contained faceted diamond nodules with a variety of morphologies and sizes, which were uniformly distributed over a large area; the average crystal size was 60nm. Reflections of cubic diamond and the bright ring was attributed to amorphous glassy carbon or diamond-like carbon. No significant scattering was attributed to graphite crystallites. 2-4nm rectangular crystallites separated by ~1nm grain boundaries were noted.
L Tang et al performed an early study in 1995 on the biocompatibility of microcrystalline diamond, and observed less cell adhesion and cell activation on diamond surfaces than on 316 stainless steel and titanium surfaces. P Bajaj et al demonstrated, using in-vitro assays, that spreading of HeLa, PC12 and MC3T3 cells was greater on ultrananocrystalline diamond than on silicon or platinum.
M Amaral et al showed that nanocrystalline diamond coatings on silicon nitride substrates exhibited higher rates of human osteoblast proliferation and matrix mineralisation than polystyrene tissue plates, while L Nordsletten et al indicated that diamond particles in cultures of human monocytes exhibited inert behaviour regarding interleukin-1 beta production and cell morphology after phagocytosis.
In 2009, A Kromka et al indicated that the surface roughness of nanocrystalline diamond coatings has a significant effect on human osteoblastic cell morphology, while in 2008 WC Clem et al demonstrated surface modification of ultra-smooth nanostructured diamond; surfaces terminated with fluorine or oxygen resisted mesenchymal stem cell adhesion. However, hydrogen-terminated surfaces enabled significant mesenchymal stem cell adhesion.
In an in-vivo study involving a rabbit model, P Aspenberg et al showed that implantation of diamond in a bone harvest chamber did not decrease bone formation, while the interaction between diamond and blood components is an important consideration when evaluating the use of this material in cardiovascular applications.
JM Garguilo et al showed that microwave plasma chemical vapour-deposited diamond films and single crystal diamond absorbed little fibrinogen. RJ Narayan et al demonstrated that nanocrystalline diamond exposed to platelet-rich plasma did not show aggregation of platelets, cells or proteins.
Implant applications
Due to their small crystallite size, nanocrystalline diamond coatings exhibit smooth surfaces. Amaral et al showed steady-state friction coefficients of 0.01-0.05 for nanocrystalline diamond in unlubricated and water-lubricated environments.
Higher friction values (0.10) were observed when testing with dilute fetal bovine serum; this was attributed to protein attachment. A high threshold load (85N) was also obtained for nanocrystalline diamond.
As a result, microcrystalline and nanocrystalline diamond coatings have potential applications in implantable cardiovascular, orthopaedic and ophthalmic devices.
Auciello et al described the use of nanocrystalline diamond in micro-electromechanical systems, fabricating a variety of MEMS, including multilevel devices and cantilevers, with ultrananocrystalline diamond. Conventional MEMS are fabricated out of silicon, which exhibits unacceptable tribological properties.
XC Xiao et al used ultrananocrystalline diamond as an inert coating for implantable retinal prostheses. They implanted ultrananocrystalline diamond in the eyes of rabbits and did not observe evidence of intraocular inflammation.
K Jozwik et al deposited a nanocrystalline diamond coating on a titanium artificial heart valve ring by means of plasma-assisted chemical vapour deposition. They demonstrated good coverage of the coating on the ring, and scanning electron microscopy and Raman spectroscopy examination of the ring after long-term mechanical fatigue testing showed that the surface remained in good condition.
MD Fries et al demonstrated microwave plasma chemical vapour deposition of titanium-aluminum-vanadium alloy structures, which were similar in geometry to temporomandibular joint prosthesis components. The uniform chemical composition of the diamond coating on the device’s condyle component was demonstrated by Raman spectroscopy.
Making sensors
Chemically modified diamond coatings can also be used in sensing and imaging applications. For example, A Haertl et al covalently attached proteins such as green fluorescent protein and an enzyme (catalase) to hydrogen-terminated nanocrystalline diamond by means of a photochemical process. JA Carlisle, meanwhile, described immobilisation of electro-active enzymes on nanocrystalline diamond coatings; these structures may be used in electrochemical biosensors.
J Rubio-Retama et al immobilised the enzyme horseradish peroxidase on the surface of nanocrystalline diamond (this electrode was used for the detection of hydrogen peroxide), and C Popov et al demonstrated attachment of ribonucleic acid molecules to nanocrystalline diamond / amorphous carbon composite films. WS Yang et al showed covalent modification of nanocrystalline diamond with deoxyribonucleic acid oligonucleotides; good selectivity and no non-specific absorption was observed. Khanna et al described the use of nanocrystalline diamond in lab-on-a-chip devices and found diamond exhibited less non-specific attachment of nucleic acid than glass, polymethylmethacrylate, polydimethylsiloxane, silicon, silicon dioxide or SU-8.
Khanna et al also fabricated microscale spikes out of nanocrystalline diamond using standard techniques, structures that were used for cell membrane puncture and cell lysis in a microfluidic device. In 2009 AM Schrand et al envisioned a variety of other biotechnology applications for nanocrystalline diamond, including use in solid phase carriers for vaccines and other pharmacologic agents.
Diamond’s commercial opportunities
Microcrystalline and nanocrystalline diamond coatings exhibit unusual chemical, mechanical and biological properties, and may be used to modify the surfaces of orthopaedic prostheses, cardiovascular devices, ophthalmic devices and microscale medical devices in the future.
Several organisations and universities are involved with fabricating and evaluating diamond-coated medical devices, and commercialisation-related challenges will need to be considered. For example, methods for scaling up the coating process are necessary in order to facilitate the commercialisation of diamond-coated medical devices. (CS Yan et al demonstrated microwave plasma chemical vapour deposition of high-quality single-crystal diamond with deposition rates between 50µm per hour and 150µm per hour.)
In addition, a reduction in the processing temperature would enable a wider variety of materials to be modified with diamond coatings. Refinement of grain size, roughness, crystallinity and other coating characteristics for each specific application is needed. Additional methodologies for reducing thermal stresses must also be created.
Diamond coatings need to become cost competitive with conventional surface modification materials. If these obstacles can be overcome, nanocrystalline and microcrystalline diamond coatings may achieve commercial importance in the medical device industry.